Into the magnetic blue yonder
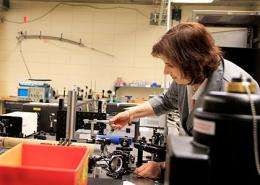
Probing the quantum mechanics of magnetism is not for the faint of heart. Literally. The door to Madalina Furis’ laboratory on the fifth floor of the Cook Building has a sign that reads “Stop! No pacemakers beyond this point.”
On the other side of the door, Furis, an assistant professor of physics, and her students, run a powerful magnet that could wreak havoc on such devices. “Sorry, but if you get a pacemaker you cannot be a high-magnetic-field researcher,” she says, with a cheerful laugh in her rich Romanian accent.
But her magnet research may help bring to life a whole new generation of devices, like computer screens that could roll up like a piece of paper.
“Making cheap, flexible electronics — that’s the ultimate goal of this work,” she says.
Furis’ research is several steps removed from manufacturing or even from materials engineering. Instead, she seeks to peek deep inside the molecular structure of strange organic compounds -- called metal phthalocyanines -- and, with a combination of light and extreme magnetic fields, catch a glimpse of certain spinning electrons.
“What we do here is probe electrons in materials,” she says. “Our field of research is to look at how electrons behave in different materials, because electrons are what makes your computer work, what makes your solid-state lighting.”
And how the electrons are arranged and flow in phthalocyanines give these materials their gorgeous blue colors (they were originally used as dyes in the early twentieth century) but also the rare property of being organic compounds that are, sometimes, magnetic.
And materials like these raise the possibility of creating semiconductors — the basis of modern electronics, from hard drives to telephones — that are made from inexpensive, flexible organic molecules. The hope is that these could be precisely engineered — “tuned,” as physicists say — to have particular combinations of magnetism and conductivity, for applications like switches and data storage.
But in order to do this, the specific families of electrons that control the degree of magnetism need to be pinpointed. Which is where very big magnets and lasers come in handy.
Magnetism has been observed since ancient times, but only in the last century have physicists exposed the underlying cause. “It’s actually the quantum mechanical properties of the electron — called spin — that is the origin of magnetization,” explains Furis.
Typically, measurements of magnetization are done in the realm of classical physics. This is the familiar, macro-scale world where, for some spooky reason, chunks of metal will stick to your refrigerator. This magnetism is measured by taking an indirect reading of an ensemble of many of these quantum-scale effects.
But that macro effect in no way points to its micro cause. “If electrons are involved in mediating this interaction, in arranging the spins, which ones of them are doing that?” asks Furis. “That’s a very big question. And that cannot be answered with a classical measurement of the net magnetization.”
Polarized probe
Getting a view of these electrons is not easy. But it turns out that photons of light — of carefully selected wavelengths and with a special twist called circular polarization — shining onto these organic materials lets Furis and her team identify and select certain electrons — some are free and some are bound to the nucleus of atoms; some are at higher energy states and others at lower ones; some line up parallel to the magnetic field, others antiparallel. And how the photons are absorbed and bounced back allows the researchers to find out which electrons are the underlying engine of that material’s magnetism.
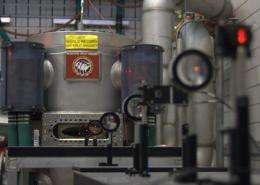
But this probing with polarized light — for materials of relatively low magnetic power like phthalocyanines — only works in the presence of a magnetic field. A very strong magnetic field.
Which is why Furis and her students place samples in the magnet here in her laboratory. Rated to five tesla (a tesla is a unit of magnetic field strength), and purchased with support from the National Science Foundation, this machine is far stronger than a typical hospital MRI machine, thousands of times stronger than the Earth’s magnetic field.
“See this?” says Lane Manning ’08, now a doctoral student in Furis’ lab. He holds up a small threaded disc with a tiny wafer of blue-green material affixed at center. It’s a sample of phthalocyanine.
“This sample is mounted on this probe here, and we’ll put the sample right in the center,” he says, screwing the disc into a large blue ring with tubes and wires sticking out in many directions. This is the UVM team’s magnet. “Now we have the sample in the magnet,” he says, “then we can bring in light to probe it.”
“And see this?” he says pointing to the opening through the middle of the magnet. “We can see through this magnet, and that is key,” he says. Instead of having to use fiber optics, this direct path “allows us to have an incredibly precise control of the polarization of the light going to the sample.” And that precision is what lets them detect the relatively weak magnetic interactions in these organic materials.
To 25 tesla
But this magnet is really just a pre-testing site, and stepping stone, to a much more powerful one that Furis and her students helped inaugurate this past summer. The new twenty-five telsa magnet at the National High Magnetic Field Laboratory at Florida State University in Tallahassee is the strongest so-called “split-magnet” in the world.
It’s hard to create a powerful magnet, but ever so much more difficult to make a window into it. But that is what this new machine does; it’s split into two halves, with a direct sightline in, which allows Furis’ team to shine polarized light at samples within, unlike other magnets of similar strength.
“Optics in high-magnetic fields were always the Cinderella of high-magnetic fields despite the huge amount of information one can get out of this,” says Furis, “because they are so hard to do.” But this machine may be ushering in a new era, she thinks.
In a first-of-its-kind experiment, with support from the National Science Foundation, Furis and several of her students, including doctoral student Zhenwen Pan and undergraduate Cody Lamarche, tested a copper-based phthalocyanine on the new Florida magnet — to see if they could identify which electrons were responsible for its magnetism.
It worked. Though the whole experiment won’t be complete until the team can return to the magnet this spring to do additional tests at extremely low temperatures, the initial results at room and fairly low temperatures were promising.
“We basically identified which electronic states are responsible for mediating this interaction,” says Furis.
Spintronics
And this kind of knowledge opens the possibility for chemists and materials engineers to “reverse engineer” desired properties into different forms of these organic semiconductors. For example, by changing the metal atom at the heart of the organic ring that forms phthalocyanines — perhaps changing it from copper to manganese — produces different numbers and densities of free electrons in the material, which, in turn, creates different magnetic strengths. Or arranging the molecules like plates in a dishrack, instead of like a vertical stack of plates, creates different patterns of electrons too.
“Almost every single device we have works because we understand the quantum mechanic properties of electrons,” says Furis.
Furis is helping lead an emerging field of physics called “spintronics” which is looking at the potential of using spin — the quantum mechanical property of electrons — instead of traditional electric charge, as means of moving and storing information.
“This work is of interest not only for organic semiconductors; there has been an effort in the world of silicon for almost twenty years to make a device where we control electron spin in the same way we control charge in transistors,” says Furis, “and having an all-organic version of that, where you could have control of charge as well as spin, would be very attractive.”
Provided by University of Vermont